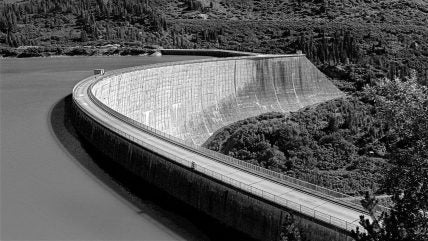
International Water Power & Dam Construction (IWP&DC), in association with Fichtner, has compiled a list of underground power houses built at some of the world’s major hydroelectric power plants.
A database containing information on 900 constructed hydropower caverns and 81 shaft power plants is presented in the following tables, with data listed by country, completion date, rock type group, dimensions, maximum excavated area and volume, rock pillar width, maximum cover height, net hydraulic head, total installed plant capacity and unit capacity, shape of cavern section, turbine type, and headrace and tailrace tunnel length. Specific excavation volumes are listed in two separate columns for plants with and without separate transformer halls.
Conventional and pumped storage power caverns and shafts have been separated into two tables since some geometry evaluations are only successful for the two singular databases, e.g. the ratio of power tunnel length (Lhead) and net pressure head (h), and typical sizes.
Compilations of hydro caverns, rock mass or rock support data have been published in previous years4,5,19,1,9,17,7,2,17,14,20,6,15,16, and similar cavern databases have been published by engineers, consultants, contractors, researchers and suppliers.
The latest edition of the hydro cavern database is a revision of an earlier compilation, produced with the support of the German Research Funding Agency, as well as Fichtner. The research was originally supervised at the Technische Universität München in Germany. Further data collected, like rock strength or support, can be obtained from previous compilations in IWP&DC or from the author*.
More than 900 caverns in 86 countries are known to be in existence today, and a further 80 cavern plants are planned for construction before the end of this decade. This implies that, on average, between 80 and 90 caverns have been constructed during each decade since 1950. However, the number of caverns envisaged may now double due to an increase in the promotion of renewable energy and private ownership.
It should be noted that by 20053 around 200 caverns had already been constructed – for example in Norway – but 110 of these caverns have not been included in this edition due to the lack of geometrical data, despite the availability of other valuable design synopsis reports8,18,13,6. This brings the total number of caverns already constructed, or planned, to a probable 1000 by the end of 2020. Power house shafts have also been published again as a separate listing.
The list of caverns published house a total of 2730 turbines, i.e. 370 x Francis, 130 x Pelton, 23 x Kaplan, and 4 x Deriaz, while another 240 conventional and 140 pumped turbine types remain unknown.
A total of 485 power tunnels and 320 tailrace tunnels, with an average length of 5000m and 1000m, respectively, are also listed. Average tailrace tunnel length for the 34 new plants included in this edition of the Yearbook increases to 1275m.
Representative hydro cavern dimensions for the 190 pumped storage plants and 610 conventional plants are 4 x 190MW, 23m x 42.5m x 123m, v = 97,000m3, and 4 x 100MW, 20m x 35.5m x 93m, v = 60,000m3, respectively:
Typical total flow rates are Q = 365m3/sec and Q = 270m3/sec for conventional and pumped storage Francis turbine plants, and Q = 40m3/sec and Q = 264m3/sec for Pelton and Kaplan turbines, respectively.
Average power shaft sizes are 190MW, d = 22, h = 55m, v = 20,000m3 for the 46 pumped storage caverns listed, and 100MW, d = 20, h = 42m, and v = 13,000m3 for the 37 conventional plants listed. These values may be biased towards larger turbine sizes since it is often only these types of projects which are reported within the hydro industry’s journals.
The 37 conventional and 46 pumped storage hydro shaft plants listed house 76 and 102 units, respectively, with an average net water head of approximately 295m.
Of the 27 and 36 listed shapes at shafts there are 20 and 23 circular shapes for conventional and pumped storage plants, respectively. Average values of 16 and 32 power tunnel lengths are similar with L = ~1800m. Typical total flow rates are Q = 260m3/sec and Q = 225m3/sec, respectively.
Currently, global installed hydropower capacity stands at 1389GW, with total installed capacity at hydro caverns taken from the published data to be at least 420,000MW, including 23,000MW at shaft power houses, 27,000MW planned for China, 26,000MW planned for India, and 32,000MW elsewhere. Construction of 80 of the 160 hydro plants planned or under construction for this decade will be in India to coincide with the country’s ongoing 50,000MW by 2020 initiative. Another 8600MW planned in Pakistan have not been included.
1. LARGEST HYDROPOWER EXCAVATIONS
To rank within the 10 largest caverns worldwide each plant needs to exceed the following: width >32m, height >74m, length >330m, section area >2000m2, excavation volume >460,000m3, total/unit capacity >4200MW/>500MW. To rank in the largest pumped storage caverns the values to exceed are: 1200MW capacity, 160,000m3 excavation volume, and flow volume of 2000m3/sec. To rank in the ten largest transformer halls a volume of 110,000m3 needs to be exceeded With regard to criteria such as cavern height, length, excavated area and volume, and total installed capacity, seven of the 10 largest caverns have either been completed already or are currently under construction in China.
The most prominent cavern plants with the largest net water head are situated in France, Italy, Peru, and Switzerland; and plants with the longest tunnel lengths are most prominent in Iceland, France, Ethiopia, Ecuador, and India.
To rank within the top ten plants with the longest tailrace tunnel lengths, 5000m and 2500m have to be exceeded for conventional and pumped storage plants, respectively.
It is also interesting to discover how frequently smaller caverns with a small total installed turbine capacity were found to be competitive by observing the 90 caverns listed with a total excavation volume of less than 20,000m3: the smallest cavern size listed is 8m x 8m x 30m; and the smallest excavation volume size is approximately 1000m3. Further small caverns are currently being prepared for construction in Greenland and Nepal, but information on their exact size is unavailable.
Keys are used to represent the prevailing host rock type, cross-section shape, and rock quality class.
2. CAVERNS IN CHINA
Total installed capacities in China, as reported in 2016, were 250GW for conventional plants, and 26GW for pumped storage plants, including semi- and fullsurface plants.
Trends in cavern sizes relating to total flow rate replace the former trend for total turbine capacity. Furthermore, plants with and without separate transformer halls are distinguished. A reduction in blast volume is significant for conventional plants. Total length, cross-section area and excavation volume are given for Francis units (sizes for 4 units) with and without separate transformer halls, respectively:
Cavern size trends for total flow volume are more reliable for larger numbers of conventional plants, and are governed by a larger number of small total flow volume plants. In contrast to the increase in cavern height there is also strong indication that caverns will decrease in width.
Total excavation volumes of pumped storage caverns, with and without separate transformer halls, does not differ much on average. The former have smaller section areas but longer lengths, and the latter have larger section areas but shorter lengths. Consequently, one linear trend for cavern volumes is sufficient.
Typical saving for other cavern dimensions, besides the strongly controlled width, is 15% if a separate transformer hall is designed.
Transformer cavern sizes were analysed for 230 plants. The volume may increase linearly with the total installed capacity:
Volume = 9000m3 + 27 * C total (MW)
Limited evaluation in Figure 4 yields in China:
Volume = 3750m3 + 37.5 * C total (MW)
The decision to construct a separate transformer cavern is becoming more frequent for larger installed turbine capacities. However, the saving is very strong for smaller power units, e.g. 15% of their dimension.
Average values for the 370 Francis and 100 Pelton turbine plants investigated are v = 83,000m3, h = 160m, C = 4 * 100MW, Q = 300m3/sec, and v = 32,000m3, h = 700m, C = 3 * 80MW, Q = 40m3/sec, respectively.
In Figure 1, and supported by reference 12, this distribution of total hydropower plant capacities shows a wide range of sizes from 200MW to 6600MW. Numerous small capacity plants may not have been adequately reported.
3. HOST ROCK FORMATIONS AT CAVERNS
Again, 165 strongly folded (katamorphic) rocks, 125 plutonic rocks, and 95 moderately folded (mesomorphic) rocks were found to be the most desirable host rocks in this ranking order. The least desirable, and more expensive to realise, were the 20 plants constructed in calcareous rocks other than limestone, and in slightly folded (epimorphic) rocks.
An effort was made to present simplified host rock formation groups from eight world regions, totalling 574, but this time disregarding power house shafts.
A further sub-division of formation rock groups is possible. The numbers from the 2015 edition of the Yearbook, including shafts, were 155 sedimentary, 285 metamorphic, and 185 magmatic formations. The almost 50% contribution of metamorphic cavern host rocks clearly prevails, apart from in China, where magmatic rock is more common.
The Yearbook sees an additional 8 caverns sited in sedimentary rock formations, 6 in metamorphic rock formations, and 10 in magmatic rock formations.
Figure 2 shows the results of a study of 46 recently constructed caverns, 49 older conventional caverns and 21 recently constructed pumped storage caverns12, and reveal that the most attractive choice are intrusive rocks, followed by calcareous rocks and, finally, effusive rocks.
Less prominent choices are clastic sediments and metamorphic rocks which are for this figure, and Figure 6, attached in the same quantities as calcareous and clastic rocks.
Throughout history the role of Europe has remained strong despite its much smaller water resources when studying the 247, 237, and 86 cavern plants in Europe, Asia, and the Americas even without considering the numerous Scandinavian plants constructed within metamorphic rocks which currently remain unreported due to the limited data available.
Some differences are noted between north and mid- America and south America where there are much more sedimentary rock formations and less metamorphic formations. Notably, much less sedimentary and more metamorphic/magmatic rock formations exist in Africa than in Asia.
The most remarkable difference between mid and south-east Asian plants, with almost 100 plants each, is 75% metamorphic but 55% magmatic rocks, respectively. Major contributions to these numbers come from two major countries, i.e. India (excluding Bhutan and Nepal) with 70-75% metamorphic formations, and China with ~52% magmatic formations and 38% sedimentary formations.
The prevailing host rock type is listed in the accompanying tables instead of combinations of formations. Average values represent preliminary evaluations of the past, and are by no means a prediction of cavern host rock formations in already distinguished regions.
4. TYPICAL ROCK PILLARS BETWEEN CAVERNS
The evaluation of Figure 3 shows the close correlation of intact rock pillar of cavern groups with the main power cavern height. A maximum value of 50m rock pillar width seems to cover all risks of unwanted structural failure modes of cavern groups even for large cavern height of ~85m. Furthermore, the cavern width seems to not be a valid criterion for the required pillar.
Considerable influences on the required rock pillar, like height, rock type and rock mass compressive strength have been discussed in previous Yearbooks. It may be concluded from the figures presented in this edition that an intact rock pillar height of 50m is a suitable asymptotic value regardless of cavern height and unfavourable rock quality, particularly within distinctly bedded and inclined rock layers.
5. GEOTECHNICAL RISKS
Geotechnical risks during cavern construction are comparable with those associated with tunnel construction, but with significantly higher impact with regard to delays and costs due to the almost impossible task of support reinforcement at higher excavation levels. In previous case histories, ‘adverse site conditions’ are seen as part of a realized risk within all possible construction risks.
Even in ‘best case’ scenarios, studies show that the number of risks increase by 40-50% during construction. As a summary of general or projectspecific investigations, the percentage observations of considerable, serious, and severe geotechnical risk impacts may be 55-65%, 15-20%, and 20-25%, respectively.
An ideal distribution for four impact grades, including disastrous risks, would be 54%, 27%, 13%, and 6% for a first attempt.
A 67% serious risk and 33% severe risk occurred due to late realisation under time pressure. Likely risk is 25%, occasional risk is 55%, and unlikely risk is 20%.
A list of adverse site conditions at approximately 50 hydro caverns was first compiled and published by Hoek & Brown in 198010, and again by Hendron & Fernandez in 19837, and were taken as starter values for the addition of projects post-1980.
The observation of adverse site conditions were taken as risks for comparison with earlier editions of the hydro cavern tables introduction. As expected, three separate rock type groups show different contributions of risk groups, and the conclusions reached in the 2015 edition of the Yearbook are repeated here, i.e.:
Sedimentary rocks are sensitive to adverse change in strong support measures, visible by the number of caverns with post-tensioned anchors and parameter variations for poor rock quality. This observation is similar in slightly metamorphic rocks. Bedded rocks are sensitive to structurally controlled cavern contour failure, clearly visible from this modelling item. Magmatic rocks are highly sensitive to the influence of lateral in situ stresses. This is also found to be similar in metamorphic rocks, which are sometimes found adjacent to them.
Adverse site conditions like high horizontal stress occur most frequently in 26% of magmatic rocks compared to 13% and 19% at the other two major rock formation groups. According to the results published in 1993 by Hibino et al8 horizontal stress factors may be up to 25% higher on average for magmatic rocks than for other formations. Modelling of site-specific in situ stresses may be required at 25% of plants, regardless of rock formation.
According to Hudson & Feng12 the typical ratio of maximum/minimum in situ stress is between 1 and 3, with an average of 1.4 for 12 and 18 layered and magmatic rock sites, respectively. The typical ratio of intact rock compressive strength and maximum cavern contour stress is between 2 and 14, with an average of 5.2, for 12 and 18 layered and magmatic rock sites, respectively.
The overall portion of caverns constructed in poor quality rock is more than 10%, and a similar quantity is observed in China.
The first 3-4 risk groups for each cavern may contain ~(2/3) and ~(6/7) of all risks, regardless of rock type. However, these results do not suggest that the two less frequent risks ~(1/7) should be disregarded.
Hudson & Feng11,12 list 14 caverns with maximum wall deformations by extreme values at seven plants, which is equivalent to 17% of the reported conventional hydro plants. Our estimate of 7.5% is therefore more than doubled and surely not biased by choice apart from the large size of turbines and caverns.
From references it may be concluded that more than 67% of the risks are geotechnical, while from site experience this is less than 40% if including all failures at construction sites.
Typical cavern risk impacts are listed, and can be divided into unacceptable, unwanted, acceptable (and insignificant) risks according to the sum of the likelihood (vertical) and impact (horizontal) number. An ideal distribution would be that the centre/ average risks should be occasional and serious (or 3+3 and clearly acceptable). However, likely and considerable is more frequent and conservative average risk grade.
6. POSSIBLE RISK MITIGATION EFFECTS
Ten simplified mitigation groups have been previously studied to distinguish between the extreme grades of very low and very high impact: These mitigation groups are as follows:
a Improved communication (or methods)
b Change order and new contract items
c Additional field investigations
d Re-analysis and re-design
e Independent second (check-) engineer
f Improved monitoring measures
g Additional laboratory investigations
h New contractor/engineer (rarely)
i Increased work capacities/resources
j Employment of specialised sub-contractor
Risk mitigation effects for 50 sites, supported by waterways risk examples and various design reports, were evaluated:
Both grades reduced 20-25% Impact is reduced 40-45% Likelihood is reduced 15-25% No grade is reduced 10-20%
Technical risk grades observed compared to contractual risks including failure at works are as follows: adverse geological changes are a higher probability but with moderate impact, and poor performance or risk of failure at the construction site create moderate probability but higher impact. The reason for this may be that for most reported case histories a geotechnical investigation was adequate and timely. In less favorable cases geotechnical risk grades may be clased as contractual.
In 2015 Hudson & Feng12 compiled a geotechnical database of 40-60 different hydro cavern examples. By evaluating these mainly large conventional hydro caverns in China several correlations/trends are supplemented:
- Total installed capacity, specific unit capacity, and unit numbers are separated for conventional and pumped storage plants.
- Simplified bedded or non-bedded or 4-5 host rock formation groups for conventional or pumped storage plants.
- Cavern group rock pillar widths and transformer cavern heights related to power cavern heights.
- Transformer cavern or surge chamber volumes and installed total plant capacity.
- Ratio of intact rock compressive strength and maximum cavern contour stress.
- Rock mass failure modes for cavern groups for bedded and non-bedded rocks.
In Figure 4 a ratio of intact rock compressive strength and maximum cavern contour stress is presented as a rough indication of local collapse safety of the designed section. Extremely low values in the range of “two” require intense monitoring. Medium values in the range of “five” are desirable. Larger values up to “14” are no design criteria but coinciding. For illustration purposes six metamorphic host rock formations are summarized either with calcareous or clastic sediments
Cover height above roof when evaluating sedimentary and magmatic formations in China shows that a larger cavern roof overburden is more acceptable for magmatic rocks than for bedded formations.
The overburden height above the cavern roof is only reported for 25 cases, along with rock formation type, and it appears that very high cover stress is more likely to be acceptable with magmatic rocks. Such information on some older conventional and pumped storage plants has not been made available.
In Figure 6 cavern rock mass failure modes are distinguished for calcareous and clastic sediments, effusive and intrusive magmatic rocks, and 10 other/ unknown rock formations. Metamorphic rock sites may be evenly distributed to calcareous and clastic sediment-based metamorphic. The remaining unknown rock formations may be possibly be magmatic rocks.
An investigation of 10 and 8 calcareous and clastic sedimentary host rocks, 10 and 8 effusive and intrusive magmatic host rocks, and ten other host rock case histories in China was carried out.
A total of 90 failure modes can be summed up in four formation groups and are roughly ~50% structural modes, ~30% other site response to excavation, and ~20% design/construction-related modes: the first group prevails for bedded rocks, the second group prevails for other rocks, and the third group prevails for magmatic rocks.
In previous editions of the International Water Power & Dam Construction Yearbook mitigations to avoid potential failures reported in China have been discussed in length, a summary of which follows:
- Immediate support reinforcement
- Design/modelling improvement
- Monitoring system reinforcement
- Risk analysis precedent application.